Laser growth of carbon fiber
We tried to use a cheap laser to grow carbon fiber directly from methane gas. It worked quickly and repeatably, but the carbon fiber wasn’t as strong as it’d need to be to make a good commercial product.
Literature from the 1990’s indicates that full-strength carbon fiber can be grown from methane or ethylene with only blue H2 as a byproduct. Since 1990’s lasers were expensive and inefficient, those fibers weren’t economical at the time, but a reanalysis with current laser costs and efficiencies indicates that laser pyrolysis could give us carbon fiber composites at perhaps 30-50% of today’s costs. Clearly that would be a nice business, and a long lever for decarbonization via vehicle lightweighting, displacement of structural metals, etc.
Sadly, when we tried it in the lab we found that 1990s claims of commercial-carbon-fiber strength from methane do not replicate; our fibers break at ~200-600MPa, generally less than 10-20% the strength of commercially available fibers. We did manage to boost their strength fivefold by passing the laser-grown fibers through a hot oven full of methane, which pyrolyzed into high-quality graphitic shells around the laser-grown cores. But the end product was too fat and rigid for textile processing, the additional pyrolysis step added cost and complexity, and the strength still fell somewhat short of our targets. So we're dropping this project for now.
Many playbooks for a green transition rely on electrified, high-temperature chemical transformations of hydrocarbons: methane pyrolysis to make hydrogen, electrified steam cracking for ethylene, etc. But so far, electricity is far more valuable than fossil heat. An electrified process that proliferates without subsidy should therefore do something that a furnace simply cannot. Electrification via laser looks appealing in this respect, as lasers can direct massive amounts of energy into tiny parcels of spacetime. The usual concern with lasers is cost (both of the capital equipment and the electricity), but these priors are due for reexamination; lasers are far cheaper than they once were. Recent growth in the laser cutting and welding sector has brought down the price of certain semiconductor laser diode systems to nearly $1/W. With an electro-optical efficiency of 60-80% and an average lifespan of over a decade, the economics of these laser diodes are akin to the now-ubiquitous LED lightbulb.
What could we make with cheap and powerful lasers? Many options come to mind. One impulse is to look to streamline and decarbonize the highest-volume and most-polluting industrial processes, as we do elsewhere[1]. But in some ways, electrifying and decarbonizing the titanic chemical commodity streams that our economy mainlines is … kind of depressing. That’s like saying “let’s reinvent the 1950s, now with a thermodynamic disadvantage”. Sometimes it’s more exciting to look for new materials that could make the future qualitatively better, or to ask what currently-exotic materials you might make abundant.
It's in that spirit that we turned to carbon fiber. With its stength (>3GPa), stiffness (>100 GPa), and light weight (<2g/cm3), carbon fiber is among the highest performance materials that grace our daily lives. Academics have been grandstanding about the potential of various nanomaterials for decades (claiming, for instance, that scaled-up carbon nanotubes would be strong enough to make a space elevator). But there’s reason to suspect that conventional carbon fiber might be about as good a structural material as carbon can be in real life, at least for human- and greater scale objects. The molecular qualities that give sp2 carbon such good in-plane strength also lead to poor adhesion between adjacent graphene sheets. That means that while CNT or graphene may have incredibly good properties at the nanoscale, they have trouble adhering in extended structures, and even a single point defect in (for example) a thousand mile-long CNT would make for a very dangerous space elevator indeed. Commercial carbon fibers, on the other hand, are graphitized from polyacrylonitrile polymer fibers. That gives you tangled ribbons of sp2 carbon on the template of the tangled polymer chains; those sheets have graphene-like (130GPa) strength on the nanoscale, but resist slipping against one another in the bulk. That’s the best way we now know to port the amazing nano-properties of carbon to everyday scales.
Unfortunately, the polyacrylonitrile (PAN) process for making carbon fibers is expensive: the material sells in bulk for $10-20/kg. So carbon fiber remains a niche material only used in cases of total necessity (helicopter blades, airplane wings) or utter frivolity (ski poles, tennis rackets… helicopter blades, airplane wings 😊).
Here’s where lasers come in. In the early 1990s, researchers used lasers to grow carbon fiber directly from methane or ethylene—with only hydrogen as a byproduct. As shown in Figure 1, Nordine et al [2] showed that by focusing a laser onto a suitable surface in the presence of methane gas, methane would locally pyrolyze to deposit solid carbon in the laser-induced hot spot (>1000 C). The deposit could be lengthened into a fiber by continually backing up the laser’s focal point as the deposit piled up. The authors claimed, tantalizingly, that these laser-grown fibers were as strong and stiff as commercial fibers produced from PAN, the expensive and energetically intensive incumbent precursor.
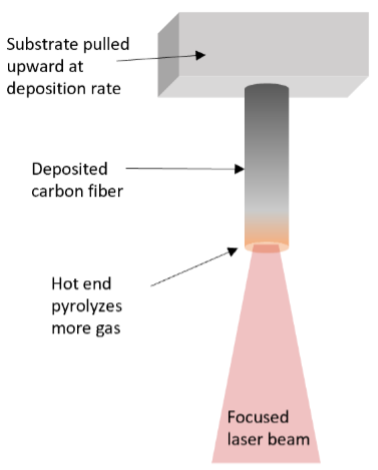
Figure 1: Cartoon of laser-grown carbon fiber process. A laser is focused to a fixed location in a chamber full of pressurized hydrocarbon gas (not shown). Carbon solids deposit on a starter substrate, which is subsequently withdrawn at the deposition rate to elongate the deposit into a fiber.
The lasers of the 1990s were far too expensive and inefficient to grow cost-competitive carbon fiber. But if useful fibers could be grown with modern semiconductor diode lasers and with the optical power inputs reported in the old literature, the laser process could cut the cost of carbon fiber substantially while reducing the total energy input per kilogram produced. The cost floor at scale would be ~$4/kg carbon material versus $15-20/kg for PAN-based fiber today. Even though existing carbon fiber markets are perhaps too small to make firm claims around CO2 emissions or drawdown potential, a laser-based carbon fiber process could make a valuable material for EVs and hydrogen infrastructure available at a lower cost and produce blue hydrogen, all in the absence of subsidy.
We tracked down Dr. Paul Nordine, the lead experimentalist of the 1990s work. Though a bit hazy on the details, Nordine delivered the validation we sought: the fiber growth worked well at lab-scale, but was abandoned for want of an efficient, low-cost laser apparatus. We designed a pressure vessel capable of laser-induced growth at up to 20 bar in methane or other hydrocarbon gases, and built an optical apparatus capable of replicating Nordine’s conditions (Figure 2).
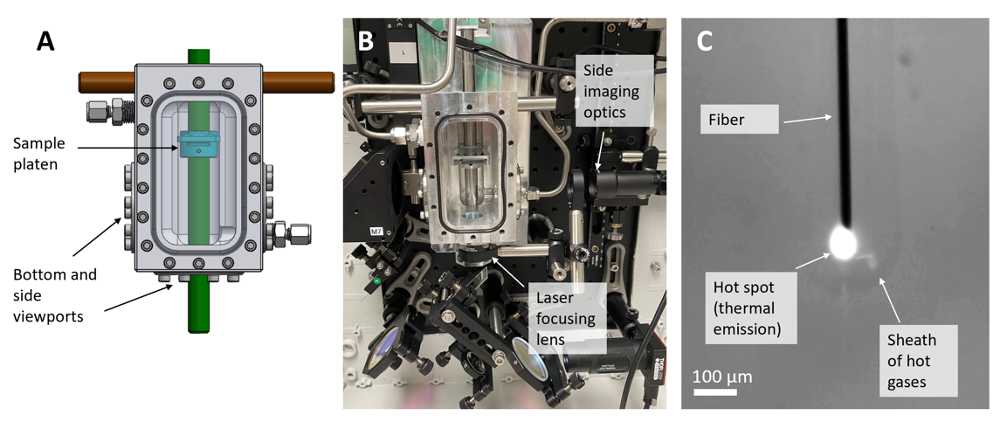
Figure 2: Experimental apparatus. (A) Model of 20 bar pressure chamber, equipped with a single-axis-translatable sample platen holding the substrate, a bottom viewport (for laser input), and two side view ports. (B) Photograph of pressure chamber mounted in optical setup. (C) View through the side port during fiber growth.The laser light (invisible in this image) is focused onto the tip of the growing fiber, creating a hot spot that emits thermal radiation. A faint sheath of hot gases can be seen rising in the chamber.
Unfortunately, while we found it possible to grow fibers of suitable diameter (8 – 30 um) at commercially interesting growth rates (0.1 – 1 mm/s) under optical powers consistent with old literature, the tensile strength and elastic modulus we measured in our lab (Figure 3) were tenfold lower than what Nordine reported as typical figures in his 1993 paper in Science[2].

Figure 3: Tensile testing apparatus (A) and example data (B). The two ends of a single fiber under test are epoxied to two aluminum plates, one of which is affixed to a table and the other to a load cell (force sensor), which in turn is mounted to a motorized stage. The stage is slowly withdrawn, straining the fiber, while the force is recorded. Our homemade fibers are about tenfold weaker (both in ultimate strength and stiffness) than commercial fibers and the figures claimed in old literature on the laser process.

Figure 4: Scanning electron micrographs of three laser-grown fibers made from ethylene under different conditions: (A) 1.2 bar and 160 mW; (B) 7.5 bar and 160 mW; (C) 7.5 bar and 250 mW. Graphitic shells are clearly visible in B and C, whereas they are less visible in A. The fibers are arranged in order of increasing Young’s modulus: 9, 23, and 28 GPa for A, B, and C, respectively.
In all, we studied about thirty fibers grown under varied parameters (power, pressure, and precursor: methane or ethylene) in the regimes identified by Nordine. The discrepancy between his claims and our mechanical measurements persists, though it’s hard to be sure exactly why. A glance at the SEM images of our fibers reveals that different morphologies are accessible in different growth regimes (Figure 4). with varying degrees of graphitization. The cylindrical shells of pyrolytic graphite seen vividly in Figure 4 (B and C) look promising at first blush, since the stiffness and strength of commercial carbon fibers is thought to derive from axially aligned graphene sheets (which themselves are among the stiffest and strongest known materials)[3]. But whereas the graphene sheets in a commercial fiber are tangled with their neighbors just enough that their nanoscale stiffness reproduces at the microscale, the constituent sheets of crystalline graphite are loosely bound and might easily slip against each other. The ordered, often paraboloidal shells in the laser-grown fibers (we like to call this a “stacked paper cups” morphology; Figure 4A and B) might furthermore facilitate crack propagation, lowering overall strength compared to the polymer-derived microstructure

Figure 5: A commercial PAN-based carbon fiber with a shell of pyrolytic graphite grown via CVD, prior to cleaving. The cleaved facet of the PAN shows a much finer, less ordered texture than the cylindrical graphite layers grown by CVD.
On the other hand, there is ample literature evidence that cylindrical graphitic shells derived from vapor-phase pyrolysis of hydrocarbons can be made comparably stiff and strong to PAN-derived carbon fibers. Work dating back to the early 1970s demonstrated that a disordered web of centimeter-scale carbon fibers could be grown via chemical vapor deposition (CVD) in a tube furnace, starting from benzene, methane, or other hydrocarbon precursors [4] [5] [6] [7]. The individual fibers isolated from this process looked microstructurally like cylindrical graphite shells several microns in diameter, and their tensile properties matched those of PAN-derived carbon fibers. (The commercial prospects of this process have evidently been limited because the tangled fibers are too porous to stand alone as a structural material, yet too impermeable to epoxies to form a reliable composite.)
Perhaps, then, the cylindrical portions of the graphite deposited via our laser process could indeed be made strong under the right growth conditions (specific combinations of temperatures, pressures, presence of particular chemical species, etc.) or post-processing (e.g., annealing). Or perhaps we could grow a shell of strong cylindrical graphite via conventional CVD on narrow laser-grown fibers. We actually tried this one in the lab; our early attempts indeed yielded clean, cylindrical graphite shells on existing fibers—both PAN-based (as a first test of the method; see Figure 5) and laser-based. Preliminary data indicate that laser-grown fibers thickened with several microns of CVD graphite can be at least twofold stiffer and stronger (tensile strength ~700 MPa) than the best fibers grown purely with the laser method.
But we also expect diminishing returns from post-growth thickening. This is partly because thick fibers can’t be formed into the woven textiles that eventually become carbon fiber composites (consider that the achievable bending radius of a standard 7-um-diameter carbon fiber is far smaller than that of, say, a hypothetical 200-um-diameter fiber). Worse, it’s often empirically found that fibers get markedly weaker as their diameter grows. This effect was documented for glass fibers as early as the 1920s and was ascribed to an increasing prevalence of surface defects induced by fabrication and handling. The 1970s carbon fiber work mentioned above[4] [5] [6] [7] found the same trend: only fibers with diameters below about ten microns had particularly remarkable tensile strength, and it dropped off rather quickly from there.
We waded into laser-based fiber growth hoping to quickly reproduce Nordine’s work. As we later learned, so did a few others [8] [9]. Everyone found very similar results, i.e., that the carbon fibers grew, but were floppy and weak. Identifying a recipe for strong laser-grown fibers continues to look like a big prize, but if there’s a secret to doing this, it’s not spelled out anywhere in the existing literature.
Many offshoots of our study are imaginable. Instead of thickening laser-based fibers, what if we thickened another weak, low-cost fiber (e.g., a carbon fiber from lignin)? Or, instead of growing fibers, our laser technology might let us 3D print graphitic structures (something along these lines has recently surfaced in the literature[10]). The fibers that we can grow are comparable in tensile strength to aluminum, even if they’re tenfold weaker than commercial carbon fibers. The thermal conductivity might approach that of graphite, which (in-plane) is fivefold more thermally conductive than copper. This suggests applications in heat sinking (e.g., for computers and batteries) or in high-temperature heat exchangers in chemical processes (graphite melts at 3600 C, and remains strong at very high temperatures). All of these seem worth investigation, but wary of an ever-expanding materials science goose chase, we’re snipping this thread for now.
Footnotes
[1] C.f. “New Frontiers for bright radiation”
[2] F. T. Wallenberger and P. C. Nordine, “Strong, Pure, and Uniform Carbon Fibers Obtained Directly from the Vapor Phase,” Science, vol. 260, no. 5104, pp. 66–68, Apr. 1993, doi: 10.1126/science.260.5104.66.
[3] X. Huang, “Fabrication and Properties of Carbon Fibers,” Materials, vol. 2, no. 4, pp. 2369–2403, Dec. 2009, doi: 10.3390/ma2042369.
[4] T. Koyama, M. Endo, and Y. Onuma, “Carbon Fibers Obtained by Thermal Decomposition of Vaporized Hydrocarbon,” Jpn. J. Appl. Phys., vol. 11, no. 4, pp. 445–449, Apr. 1972, doi: 10.1143/JJAP.11.445.
[5] T. Koyama, M. Endo, and Y. Hishiyama, “Structure and Properties of Graphitized Carbon Fiber,” Jpn. J. Appl. Phys., vol. 13, no. 12, pp. 1933–1939, Dec. 1974, doi: 10.1143/JJAP.13.1933.
[6] G. G. Tibbetts and C. P. Beetz, “Mechanical properties of vapour-grown carbon fibres,” J. Phys. D: Appl. Phys., vol. 20, no. 3, pp. 292–297, Mar. 1987, doi: 10.1088/0022-3727/20/3/008.
[7] C. Sauder, J. Lamon, and R. Pailler, “The tensile properties of carbon matrices at temperatures up to 2200°C,” Carbon, vol. 43, no. 10, pp. 2054–2065, Aug. 2005, doi: 10.1016/j.carbon.2005.03.020.
[8] J. L. Rife et al. “Structural and mechanical characterization of carbon fibers grown by laser induced chemical vapor deposition at hyperbaric pressures,” Carbon, vol. 162, pp. 95-105, June 2020, doi: 10.1016/j.carbon.2020.02.018
[9] C. A. Cook, G. B. Thompson, “Characteristics of methane and ethylene on additively deposited carbon fibers,” Carbon Trends, vol. 11, 100258, June 2023, doi: 10.1016/j.cartre.2023.100258
One reason we're starting to put some of these notes online is in the hope that people can Google around and avoid doing too many more Nordine replications, or other unneccessary repeated work.
[10] F.E. Torres-Davila, K.L. Chagoya, E.E. Blanco et al. “Room temperature 3D carbon microprinting,” Nat. Commun., no. 15, 2745 (2024), doi: 10.1038/s41467-024-47076-z