Checking some prejudices on materials decarbonization
We'll make more decarbonization progress by inventing the materials of the future than by reinventing the materials of the past.
To suggest new carbon-free ways of making old materials like cement, steel, etc. can feel like saying “let’s reinvent the materials economy of the 1950s, now with a thermodynamic disadvantage”. That’s not a vision of the future at all—and it probably won’t beat the old system on cost. Instead, let’s use new electrochemical and biosynthetic techniques to make high performance materials that actually feel like the future, and whose adoption could cut carbon too.
Decarbonization isn’t just about a shift in energy sources. As you probably know, decarbonization also portends enormous changes in how we use energy to make things.
The old way of making things was thermochemical. Since fossil carbon is both a good source of heat and a good chemical reducing agent, our legacy chemical processes often resemble explosions that have been cleverly frozen at various stages to make the products we want. Cement, steel, plastic, fertilizer [1]—and so much else. Think of the dirty roar of a giant steam reformer, chemical cracker, or blast furnace.
Judging by all the new projects out there in academia, industry and the startup world, the new ways of making things seem to be either electrochemical or biological. Electrochemistry for inorganic materials, synbio for organic ones. These are the most obvious ways to use green electricity or agricultural feedstocks to make the products we get from fossil energy now. Think of the quiet power of an electrolyzer, or the bubbling of a fermentation vat [2].
So while the explicit assumption of a net-zero materials economy is that baseload green electricity or truly sustainable biofeedstocks will become widely available and affordable, an implicit assumption is that these very different methods of materials production can someday mature as drop-in replacements for the processes we run now.
But is that right? In my opinion, there are reasons to doubt that electrochemical or biological processes can sub in for thermochemistry so easily. All else being equal, my priors say a thermochemical process will beat an electrochemical one at scale; a reaction in a volume is effectively “3D” while one on an electrode surface is “2D”—hence the thermochemical process has a power law advantage when it comes to reaction rate per capital expense. I have similar skepticism about bioprocess scaling. Biology happens in water, at mild temperatures, at neutral pH, while chemical processes can happen at extremes of temperature, pressure, equilibrium constant—whatever it takes to make the product cheaply and quickly. Of course thermochemistry will win.
But those are just prejudices! To interrogate them, let’s look at some data on the balance between thermochemical, electrochemical, and biological production methods for the materials we use today.
Electrochemistry vs thermochemistry
To get specific, my prior was that all else being equal a thermochemical process will win on scale (3D vs 2D equipment), but that electrochemistry can win in specific cases where there are coproducts (like chlorine production) or very reducing potentials (like aluminum production) [3].
My Orca colleague Aravindh and I decided the best way to stress-test that assumption was to make a map of all major inorganic chemicals, sorted by the energy of the reaction (in voltage, energy per electron) and value per energy (i.e. what fraction of the value of the commodity is its embodied energy). The dot sizes are market volumes in tons per year on a log scale.
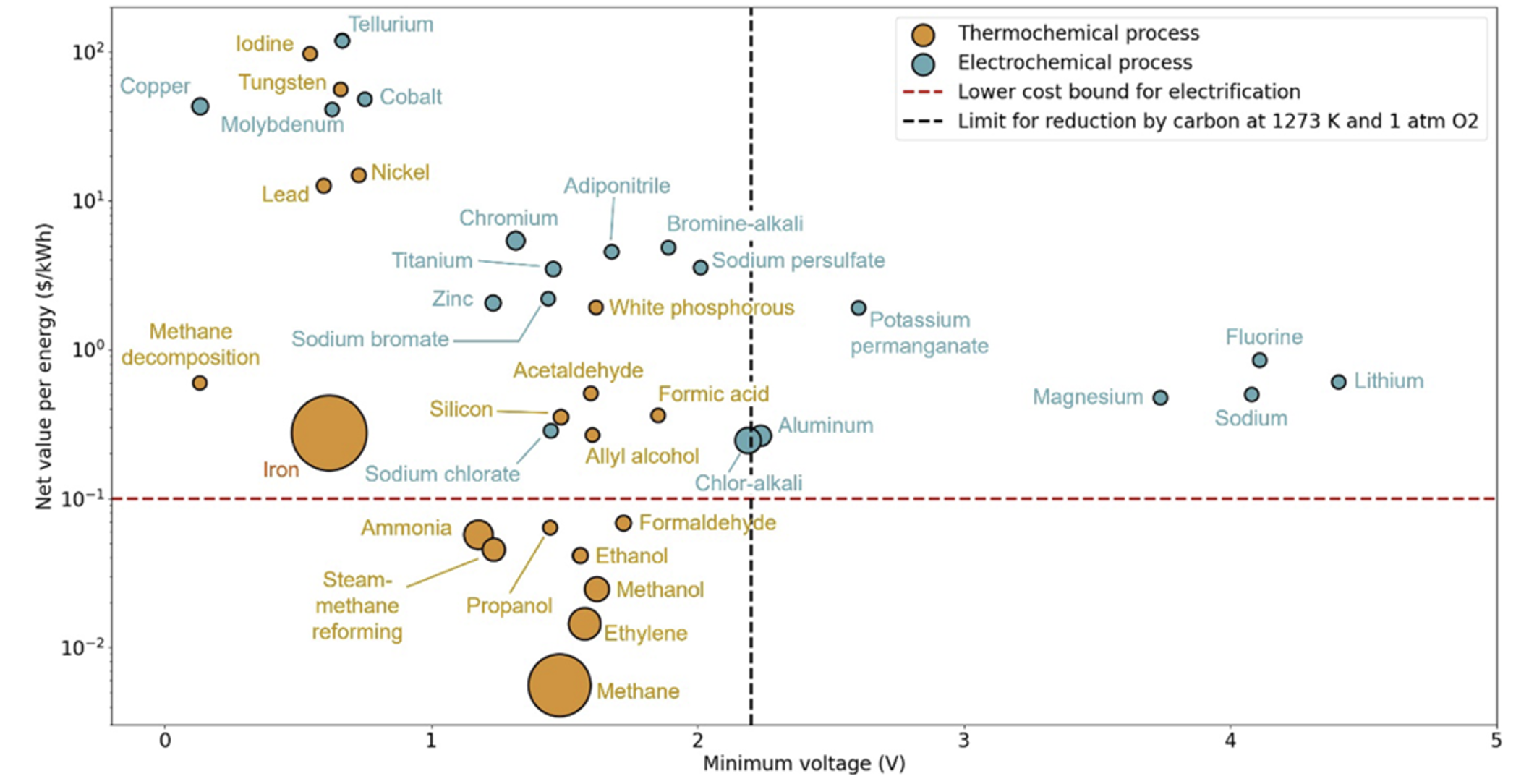
We dropped a horizontal line on the plot to denote the lower bound of feasibility for electrification based on electricity prices. We also dropped a vertical line to show the limit of what you can reduce with carbon up to 1000ºC. That gives a plot with four quadrants.
In the top right quadrant, electrochemistry is basically obligatory. You can’t reach those potentials with carbon as a reducing agent, and every single commodity there is already produced electrochemically. In this region, the energy transition is ready to go. We’re just waiting on the clean electricity [4]
In the bottom left quadrant, fossil-powered thermochemistry is universal for energy cost reasons. Electricity is not cheap enough, and electrochemical reactors are at additional disadvantage from scaling. These processes will be very hard to decarbonize without substantial subsidy.
The top left quadrant is where it gets more interesting. Both thermochemistry and electrochemistry coexist, but nearly every extant electrochemical example brings in a secondary advantage like purification (e.g. Cu), surface sensitivity (e.g. galvanization), a very oxidizing potential (persulfate), or valuable coproducts (chlor-alkali). Basically, electrochemistry needs to serve as more than a mere method of reduction to compete in this region [5] .
Biology vs. chemistry
My prior here was that biology wins at making complex compounds, chemistry wins at making simple things cheaply. This view came from looking at how things like the amino acids are produced; where chirality doesn’t matter (glycine and methionine) they’re made chemically, where it does (all the rest) they’re made either biologically or semi-biologically with immobilized enzymes.
What happens when you shake out that prejudice by looking at the whole chemical industry? My colleague Mahati looked at the top 70ish organic chemicals produced worldwide by market value:
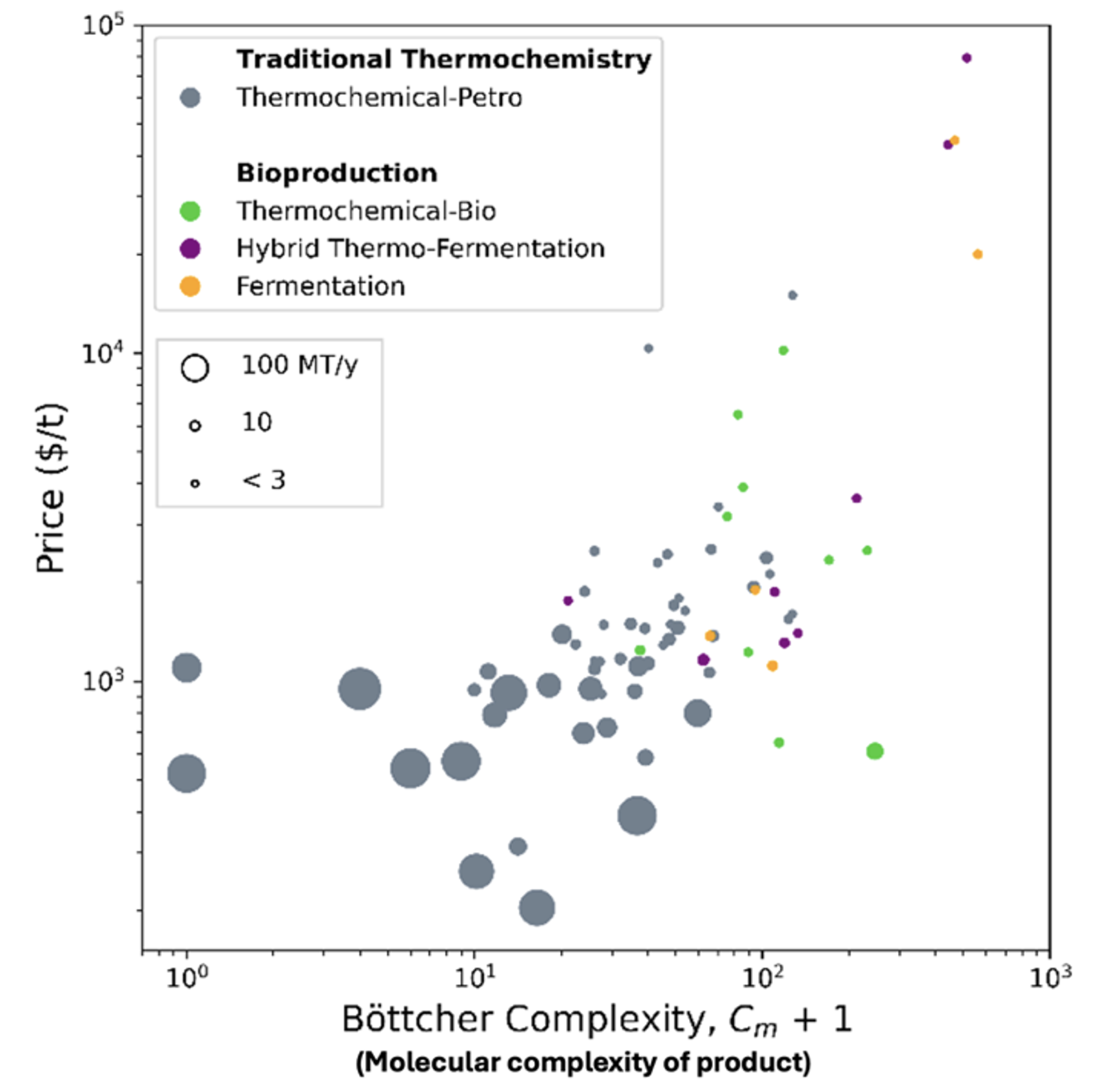
Mahati plotted them here based on price per ton and on the chemical complexity (a metric called Böttcher that counts number of atoms, heteroatoms, chiral centers etc.; ethylene is complexity of 2, propylene is 4 and e.g. doxycycline is >100). Grey dots represent thermochemical production using petrochemical feedstock, green represent thermochemical transformations of agricultural feedstock and so forth. Dots are again sized to the world market size.
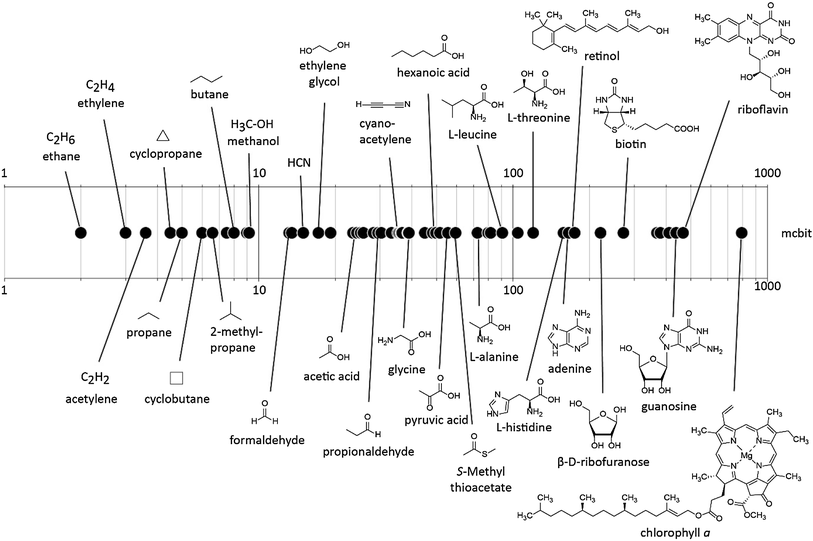
The trend is pretty clear:
- Chemical techniques blast systems out of equilibrium using extremes of pressure, temperature, and chemical potential. That gives you high reaction and conversion rates, but relatively little control over exactly which bonds you make or break. Think of e.g. ethylene production, a reactor bigger than a house with gas moving through it at almost the speed of sound [6] .
- Biology works near room temperature and in liquid water. Action potentials tend to be small multiples of KbT, and chemical conditions tend to be mild to avoid harming biochemical machinery—that means processes tend to be near equilibrium, and reactions go slower. But it also means that complicated chemical systems can operate with far more finesse. Think of assembling and folding proteins into amazing molecular machines, like the jaws of nitrogenase or the turbine of ATP synthase.
Chemistry has a cost advantage for making simple stuff, but it vanishes quickly for making more complicated products. Another way to look at it is that chemical techniques are better at changing between energy states, biological techniques are better at changing between complexity states. Another representation of the top 70 commodity chemicals shows this clearly:

Thermochemical techniques (again gray) give enthalpy (ΔΗ) changes cheaply; complexity (Cm) changes come dearly. Bioprocesses are the opposite [7].
It’s possible that recent advances in synthetic biology change how this situation is polarized. But I suspect the opposite. After all, synbio techniques are mostly techniques for giving bugs much more precise instructions for what to make, not some kind of revolution in their basic metabolism. So there’s every reason to think synbio advances will specialize bioproccesing techinques even further away from energetics, and even more towards complexity.
Wrapping up
My prejudices appear to be basically correct.
Of course, they’re still prejudices. A genuinely new technical approach could certainly break all of the scaling relations we see in the economy today [8] .
But overall I remain darkly skeptical of synthetic biology approaches to making the big important climate-relevant chemicals, which tend to be simple compounds. Or proposals to blithely electrify the big ugly processes like cement or steel using electrolysis when secondary advantages like purity or coproducts aren’t on offer.
Where does this leave us--can electrochemistry or synthetic biology help us with decarbonization at all? Is there a positive lesson to take from these cruel stereotypes? I think so.
The hardest to decarbonize materials are hard to decarbonize first and foremost because they’re dirt cheap. But you might also notice that many of them aren’t particularly cool materials. Cement and steel and plastic. That's not what I'd want to build the future out of.
In some ways, electrifying and decarbonizing the titanic chemical commodity streams that have built our world since the 1940s is a pretty bleak picture of the future. Cement and steel and plastic are the materials for quantity, not for quality. In the future, shouldn’t we have both? It’s one thing to decarbonize the rust belt, but does it still have to rust? What about better materials?
Consider the light metals in the top right quadrant in the electrochemistry figure. Al and Mg both have far higher strength/weight ratios than steel; they resist corrosion much better [9]; their production is already electrified; and their ores are arguably easier to find– Al is the most common metal in the earth’s crust, Mg is extractable directly from seawater. Both metals are more expensive than Fe today, primarily because they take much more energy to produce. But in a world with cheaper clean electricity – the world we need if we want to decarbonize steel production anyway—in the future we want, we should expect more use of Al and Mg, and much less use of steel[10].

As you can see with these 'idiot indices' (cost of metal divided by cost of ore plus energy of reduction), these light metals have vastly more room to come down in cost too. So why are hundreds of startups looking to electrify steel production, but you can count the enhanced Ti or Mg projects on the fingers of one hand [11]?
That’s where all of these prejudices point, in my opinion. To try to get electrochemical or synbio techniques to sub in for ancient thermochemistry is like saying “let’s reinvent the 1950s, except more electrical and more cellular”. That’s hardly a vision for the future at all. Instead, let’s be honest about where these techniques can actually shine, and then use them to build a future out of the materials that we really want.
Footnotes
[1] Vaclav Smil, Reliance on fossil fuels
[2] It’s hard to overstate how widespread this is. When researchers and climate entrepreneurs want to replace a polluting inorganic chemical, the tool they reach for is electrochemistry. Electrochemical cement, steel, ammonia, hydrogen etc. And when they want to decarbonize an organic chemical, the tool they reach for first is nearly always synthetic biology. Bio-ethanol, bio-plastics, biofuels etc.
[3] Fossil carbon provides a means of reduction, which combined with our planet’s oxidizing atmosphere makes for a convenient source of energy. Electrochemistry always couples both an oxidation and a reduction reaction with a separation from your electrolyte—ideally, you can find value in all three parts.
[4] It’s worth noting that this chart is systematically favorable to electrochemistry. We colored processes blue if the commodities were produced electrochemically to any appreciable extent. For example Mg is made electrochemically in the U.S., and thermochemically in China (possible because you can pull vacuum and remove Mg as vapor). And Ti is reduced using Mg. Both are ‘electrochemical’ on our plot.
[5] Over time humanity has been marching rightward across this graph, using materials that take more and more energy to wrest from their ores
>6000 years ago we were at 0V. That’s the stone age, using oxides directly as tools.
6000 years ago we were at 0.13V. That’s the copper age, copper requires smelting, but not particularly high energy, 0.13V from sulfide ore, 0.4V vs oxide ore
5000 years ago we were up to 0.6-0.7 V. That’s the bronze age, an eighth part tin in copper.
3000 years ago we got up into the 0.6-1V range, the iron age.
You might guess that as energy becomes more abundant, we will continue this rightward march towards Al, Mg, and Ti.
One thing that would blow up this whole view of world progress: cheap geologic H2. Planet earth tends to be acidic and oxidizing on the outside, basic and reducing on the inside. Those potential gradients have for all human history been basically unreachable to us, blocked by many miles of rock. But if the universe’s most mobile molecule is indeed capable of connecting those two worlds, all of this would change, and huge amounts of nonpolluting energy would suddenly be accessible.
At least one startup company claims large scale geologic H2 for <$1/kg and <0.5kg CO2/kg H2. If that’s true and if it scales, the story of decarbonization would no longer be about electrification. H2 after all is a chemical reducing agent. Everything on the left half of the plot would be made with H2. Suddenly our worldview would flip over the y-axis and those would be the easiest to decarbonize commodities.
[6] The fuel pumps on the Saturn V rocket engine were 40MW. The pumps on an ethylene cracker can be >100MW. The first step in plastic production is like a giant rocket blasting into a tube, precisely controlled, all day every day.
[7] These plots drop ethanol for simplicity (it’s the largest commodity produced in quantity from both agricultural and fossil feedstocks.
[8] I’d count plasma chemistry, photochemistry, and even electrically-powered thermochemistry as approaches that you can’t discount based on these observations, though all are too expensive for the time being.
[9] Incredibly, Fe corrosion alone is thought to cost the world several percent of GDP.
[10]Every proposal we hear for electric steel seems to assume free or near-free green electrons. That includes electrolytic hydrogen direct reduction, electroreduction etc.
But that makes me wonder, if electrons truly get cheap enough to make green steel economical, might we just switch to Al for our structural material of choice instead? Very rough numbers:
Fe: $0.9/kg now (2/3 ore, 1/6th energy, 1/6th other)
Al: $2.20/kg now (1/3 electricity, 1/3 carbon, 1/3 ore+heat+NaOH)
Say the Alcoa carbon-free anode process is used, Al cost is now 2/3 electricity. Also say that green electricity becomes basically free. Now iron is $0.72/kg. Al would be $0.69/kg. Factor in its far higher strength to weight ratio (a small mass of Al can replace a large mass of steel), its corrosion resistance and recycleability, and it seems like you'd replace a lot of structural steel.
In reality, the substitution of Al for steel might not go that far, and it might take a long time. Strength-to-weight isn't everything. But it does make current decarbonization efforts that focus almost-exclusively on steel look even more lopsided.
[11] There are reasons to applaud aspects of subsidy programs like the Inflation Reduction Act, but this points to a liability. They can be prescriptive.
Gov’t money for science makes sense. Progress in science is basically cumulative. Different answers to a scientific question tend to be mutually illuminating. But subsidies towards particular engineering approaches? “Progress” in engineering can go backwards. If a subsidy deepens a local equilibrium, economically speaking, it could make it harder to reach a global equilibrium that might be better overall.
What if the local equilibrium has us using steel as the world’s main structural material for now, but with innovation and an unsubsidized energy transition the global equilibrium would show Al, Mg, or carbon fiber as the structural material of the future? Subsidies for green steel will make that new future harder to achieve. Similar arguments can be made for hydrogen, thermal storage, battery storage—nearly all of it. There’s a risk that the IRA could lock us into the material economy of the 1950s for a long time.